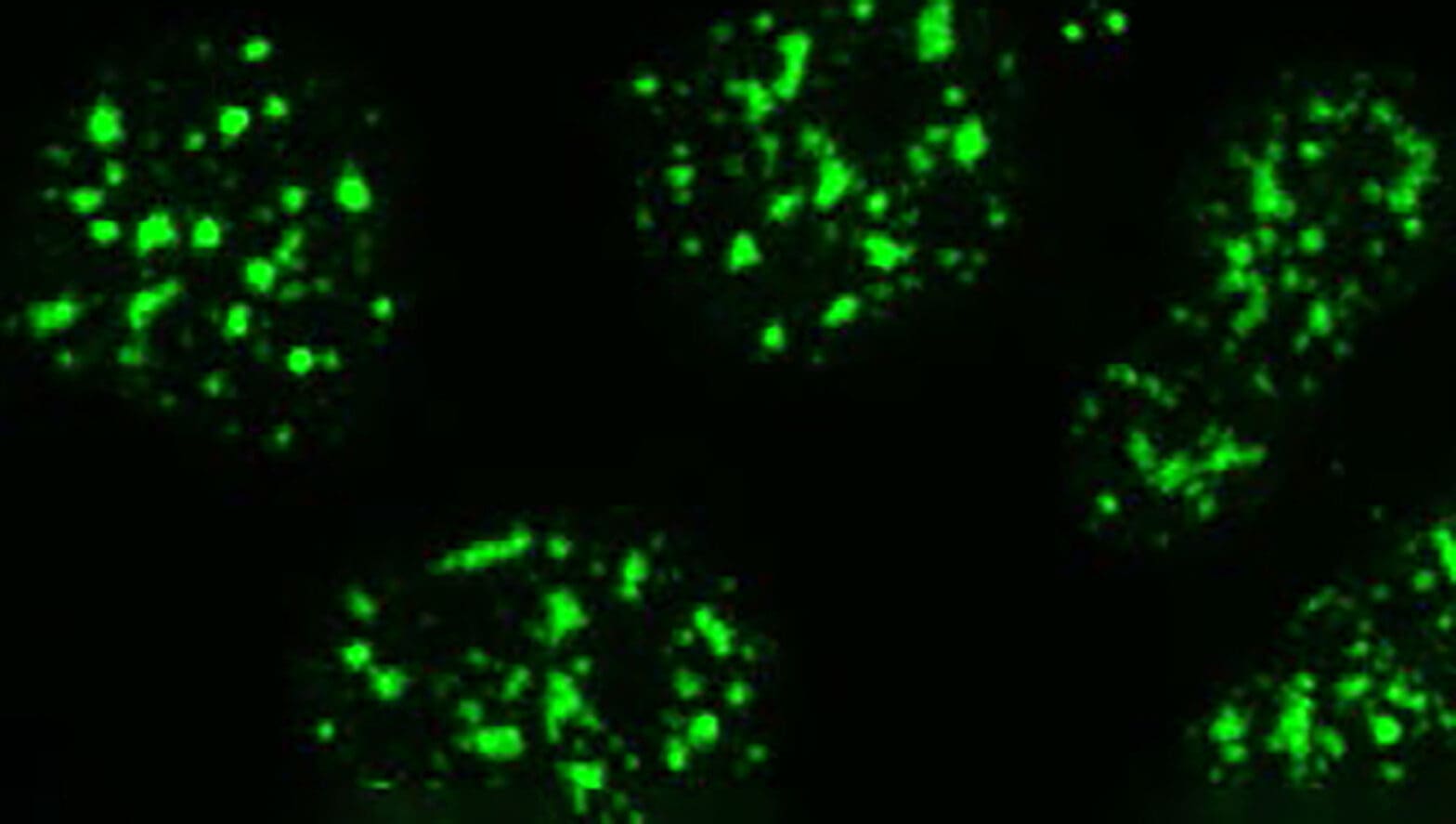
Bioengineers solved a mystery behind cellular droplets with a view to future research and medical treatment
Now, researchers led by scientists at Princeton have uncovered a key process underlying condensates’ development. The research also explains why drops appear in a wide range of sizes and numbers.
In the study, published this month in Nature Physics, the Princeton-led team showed that condensates develop through two competing processes. One process, nucleation, refers to the initial formation of droplets inside cells. The other, coalescence, occurs when drifting droplets collide and glom together into bigger condensates.
Together, the processes proceed in mathematically predictable ways. The findings will help researchers track condensate development, potentially leading to novel medical therapies and biotechnology applications.
“With this study, we have successfully identified the underlying processes that give rise to the sizes and numbers of condensates observed in living cells,” said Ned Wingreen, the Howard A. Prior Professor in the Life Sciences who led the research along with Clifford Brangwynne, the June K. Wu ’92 Professor of Chemical and Biological Engineering. “Despite the very complex environments inside cells, we have been able to elucidate simple mathematical properties and principles about condensate growth and size distribution.”
Mapping the mechanisms behind condensate development could prove especially insightful for treating neurological conditions such as Alzheimer’s disease, Huntington’s disease, and amyotrophic lateral sclerosis (Lou Gehrig’s disease). Abnormally large and partially solid-like droplets formed around protein clumps have been linked to such disorders.
“This study is important for establishing a quantitative framework for understanding the fundamental physical mechanism behind protein aggregation, a necessary step for developing treatments for a range of devastating diseases,” said Brangwynne.
The lead author of the paper, Daniel Lee, now a postdoctoral fellow at the University of California, Berkeley, conducted the research as a graduate student at Princeton with Brangwynne and Wingreen. Lee and colleagues performed experiments with live cells and synthetic condensates, and additionally ran computer simulations to develop and test theoretical models.
The lab work consisted of tagging proteins in condensates with light-sensitive markers, enabling the researchers to observe and track the condensates’ growth and behaviors. Tools developed in Brangwynne’s lab allowed the researchers to shine light at specially tagged biomolecules and trigger condensate formation.
In parallel, the researchers specifically examined natural condensates as well as those associated with Huntington’s disease. First, the authors measured the size distribution of natural condensates called nuclear speckles—miniscule blobs that emerge in the cell’s nucleus and which house proteins and RNA. The experiment showed that nucleation of these small condensates occurs rapidly in the nucleus, followed by a slower process of coalescence when the moving droplets bump into each other and merge. By contrast, the proteins associated with Huntington’s disease nucleated continuously. Because bigger condensates have greater odds of encountering other droplets, in this case the biggest condensates tended to keep getting bigger by sweeping up the small newly formed ones. This “rich get richer” behavior means that only a small number of very large condensates develop over time in a given cellular environment. Mathematically, this stratification of sizes followed a so-called power law distribution. Such laws are common in nature. An example is that while ants are extremely small compared to the largest animals, ants also are extremely abundant compared to those largest animals.
“We saw that the Huntington’s disease condensates grow by scooping up or ‘snowballing’ material as the new material that can form condensates is constantly produced in cells,” says Wingreen. “But condensates do not all grow at the same rates because the bigger condensates take up correspondingly more of the highly abundant, continuously produced little condensates. These mathematical relationships are simple, describable, and beautiful.”
David Zwicker, a theoretical physicist who studies the organization of biological soft matter at the Max Planck Institute for Dynamics and Self-Organization in Germany, said the experiments answered important questions about the process cells use to control droplets.
“Intriguingly, they find significant differences between size distributions of native and pathological condensates, suggesting that they are controlled by qualitatively different processes,” said Zwicker, who was not involved in the research. “Understanding these differences in detail will help us to identify and potentially affect pathologies involving condensates in the future.”
Looking ahead, the researchers plan to build on their findings to learn more about controlling the sizes of condensates in cells. Condensate function appears closely related to size, meaning that modulating size distributions in cells could help those cells perform metabolic tasks more effectively.
“In many cases, there is a sweet spot for condensate size—not too small, not too large—where function is optimized,” Wingreen says. “Being able to control that sizing would open up very promising avenues for new therapies and biotechnologies.”
In medicine, regulating condensate size with drugs could treat diseases where condensate size distributions have gone awry and gum up a cell’s works by forming aggregates of material. Meanwhile, in biotechnology, scientists could engineer bacterial and fungal cells to produce fuel products for energy generation, for instance, or to break down industrial toxins for environmental remediation.
“There is a lot of excitement about condensates in many fields of science right now,” says Wingreen. “We’re only starting to wrap our heads around the underlying biology and dynamics of these amazing droplets.”
Besides Brangwynne, Wingreen and Lee, authors include: Chang-Hyun Choi, graduate student, and David W. Sanders, associate researcher scholar, the Department of Chemical and Biological Engineering at Princeton; Lien Beckers of Princeton and the Howard Hughes Medical Institute; and Joshua Riback, a Princeton postdoctoral fellow now on the faculty at Baylor College of Medicine. The work was supported in part by the National Institutes of Health, the Howard Hughes Medical Institute, and the National Science Foundation.